About our Research
The Zia lab is a multi-disciplinary team tackling big questions and solving problems at the interface of physics and biology using scientific computing. We are currently focused on harnessing the Brownian physics in biological cells that regulate their response to the environment, establishing mechanisms of complexification that transform matter to life, and hardwiring their genetic programming to resist deleterious mutations. We also engineer non-living suspensions, collaborating with experimentalists to control molecular scale interactions between proteins to enhance monoclonal antibodies, gel stability, and more.
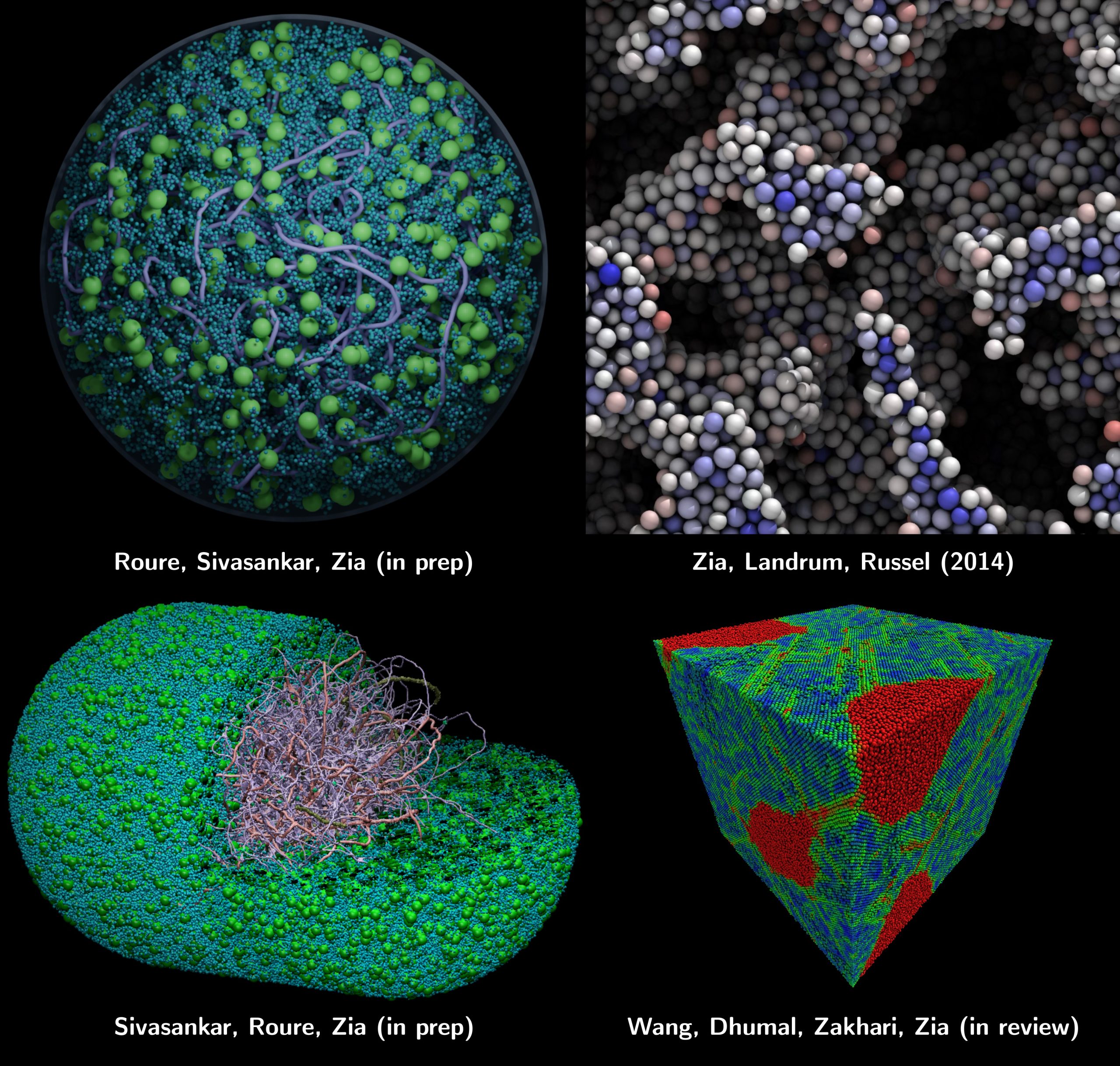
Research Topics
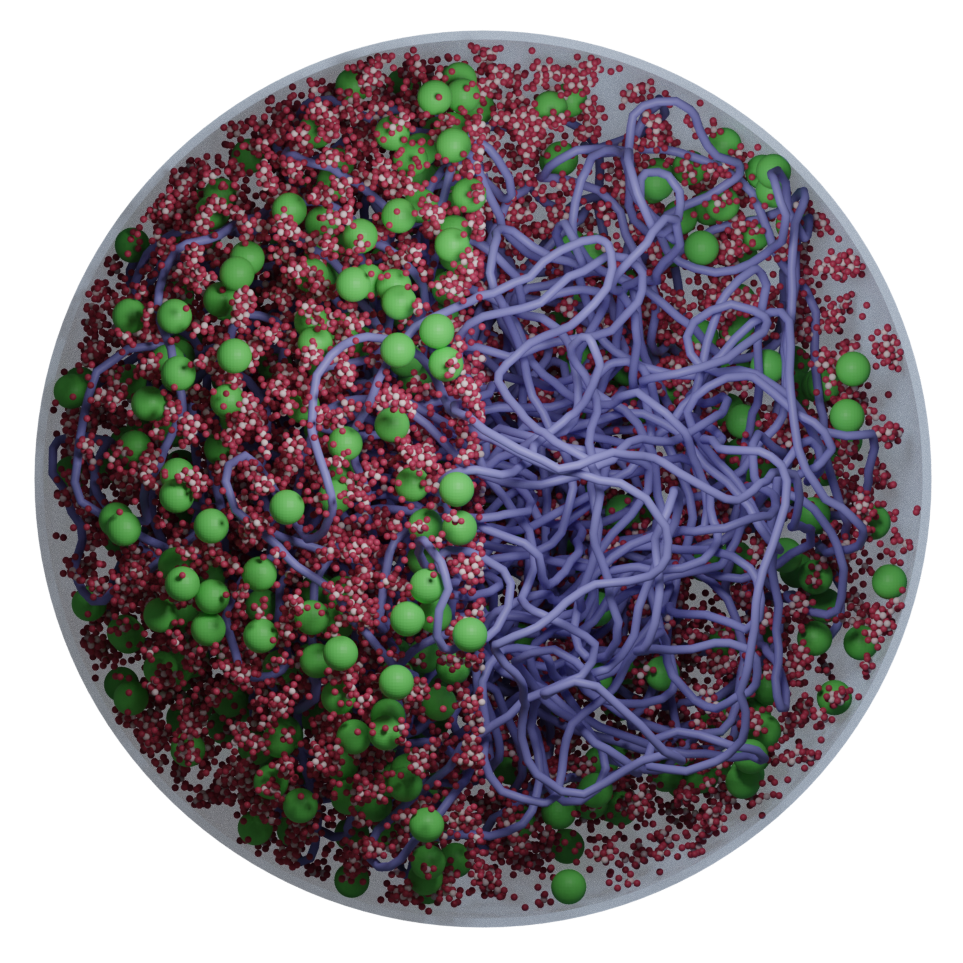
Whole-cell models of bacteria
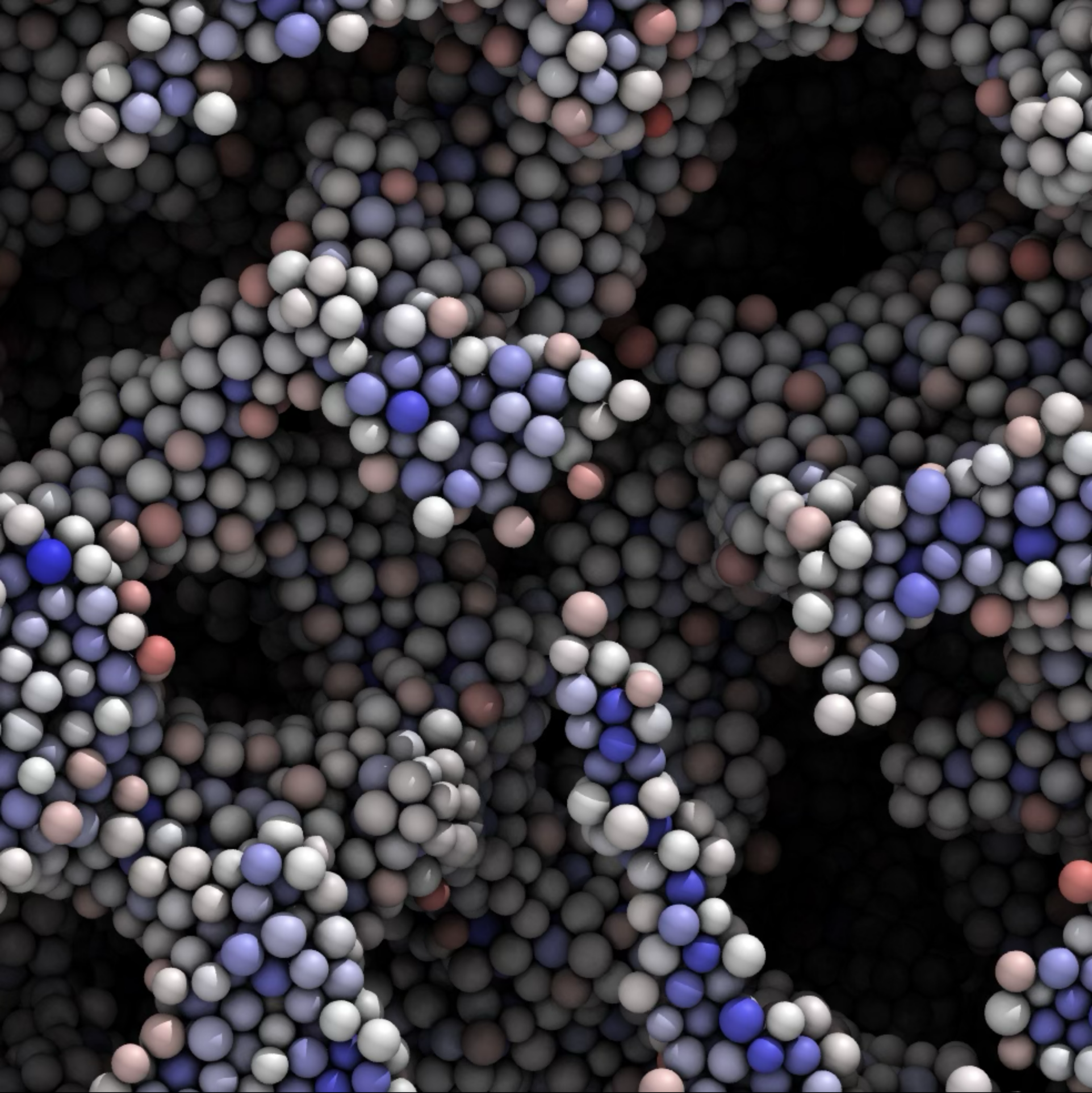
Proteins and other colloidal gels
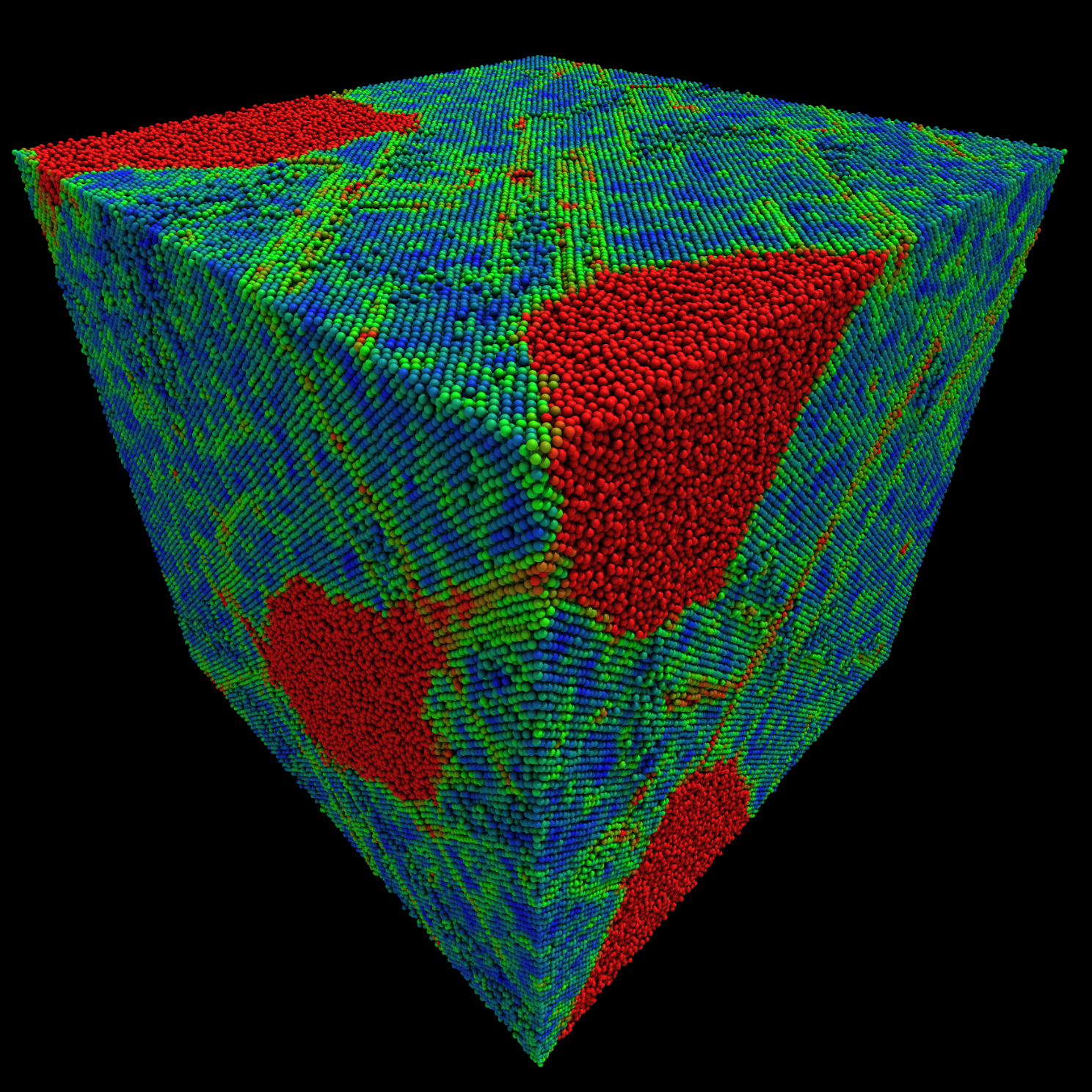
Colloidal crystals and glasses
Whole-cell models of bacteria
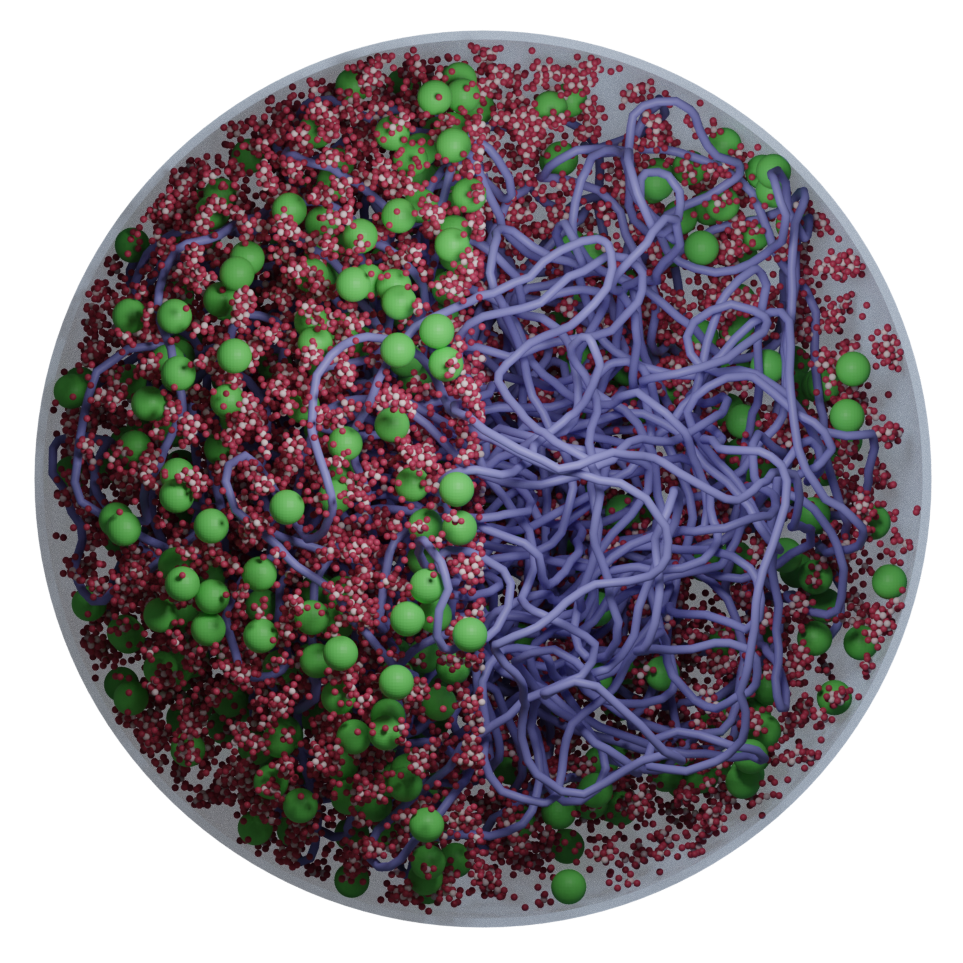
Engineering biological cells is at the forefront of cancer treatment, gene therapy, biofilm control, bio-defense, and more. Understanding of how cells operate can lead to tools and technologies that broadly advance such research. There are 3 approaches to whole-cell modeling: kinetics-only models that mimic entire cell life cycles but abstract away all physical context, missing crucial phenomena such as condensates. Nearly all-atom models attempt total resolution but even a few reactions would take years of global compute power. Our lab’s whole-cell bacteria models use fast colloidal-scale refining to capture high resolution with minimal computational complexity.
- Focus area 1: The physics of life: modeling JCVI-Syn3A
- Focus area 2: Mutation control: engineering E. coli
- Focus area 3: Coarse graining: residue-level interactions in cytoplasm
The physics of life: modeling JCVI-Syn3A
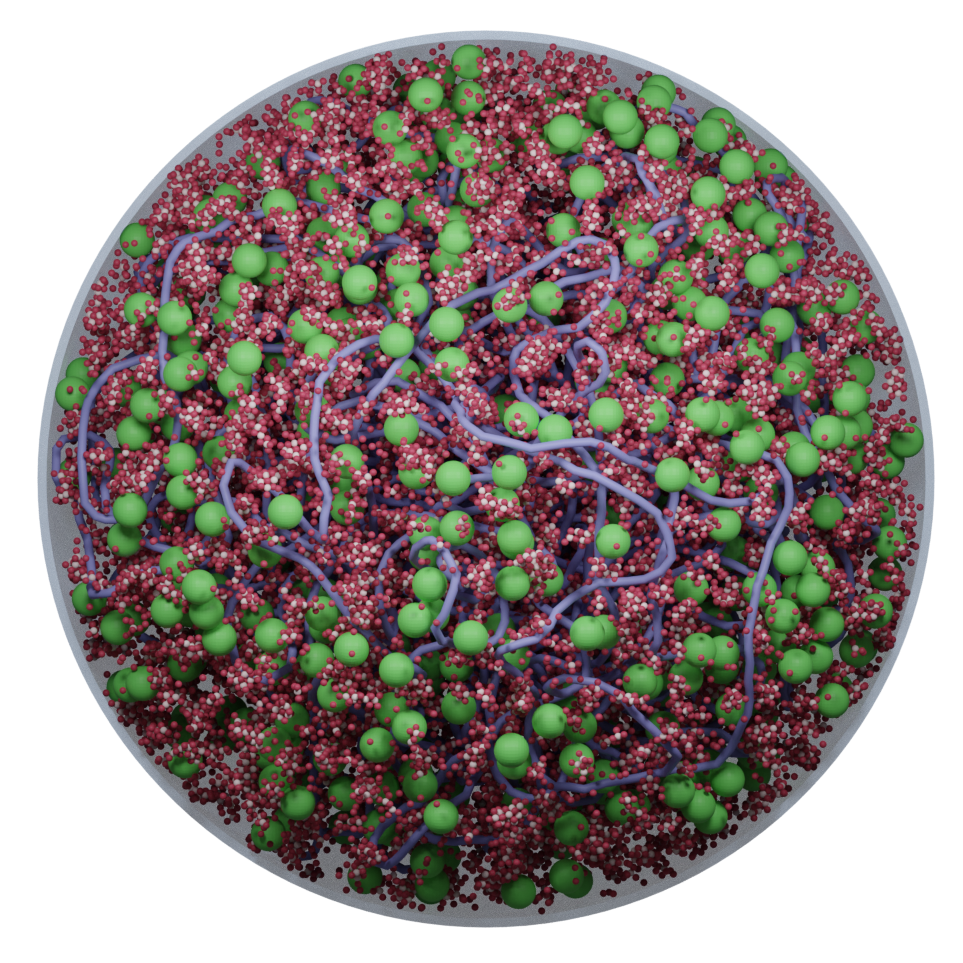
Our lab searches for how physical principles produce the basic elements of life in biological cells: growth, replication, and heredity. A cell comprising the minimal set of genes essential to life is an ideal proving ground for probing this matter/life nexus. This minimal system is encompassed in a well-known cell with the simplest naturally occurring genome, Mycoplasma genitalium. Hutchison, Glass, Venter, and co-workers reduced this to the smallest genome capable of life in the JCVI-Syn3A synthetic cell. Our models of this cell are demonstrating the specific physics that lead matter to life. Potential practical outcomes include new opportunities to design bespoke genomes. Next area
“Physiotype” to phenotype: engineering E. coli
Bacterial adaptation and mutation drive antibiotic resistance, degrade bacteria-based drugs, and erase diagnostic markers. Insufficient mechanistic understanding of this response limits practical countermeasures. We propose that physical DNA remodeling that undergirds such adaptations can be durably controlled using physical constraints. The objective of this work is to identify key motifs of the bacterial nucleoid, which we call “physiotypes”, as potential targets for such control. To do so, we will use our whole-cell computational models of E. coli to mimic several key nucleoid remodeling responses to environmental triggers. Outcomes will include modeling tools for biotherapeutics and improved physically-resolved models of growing and dividing cells. Next area
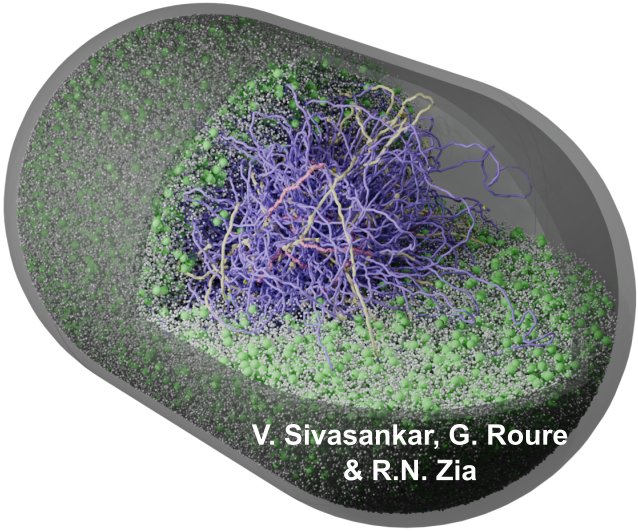
Residue-level interactions in whole-cell cytoplasm: algorithms
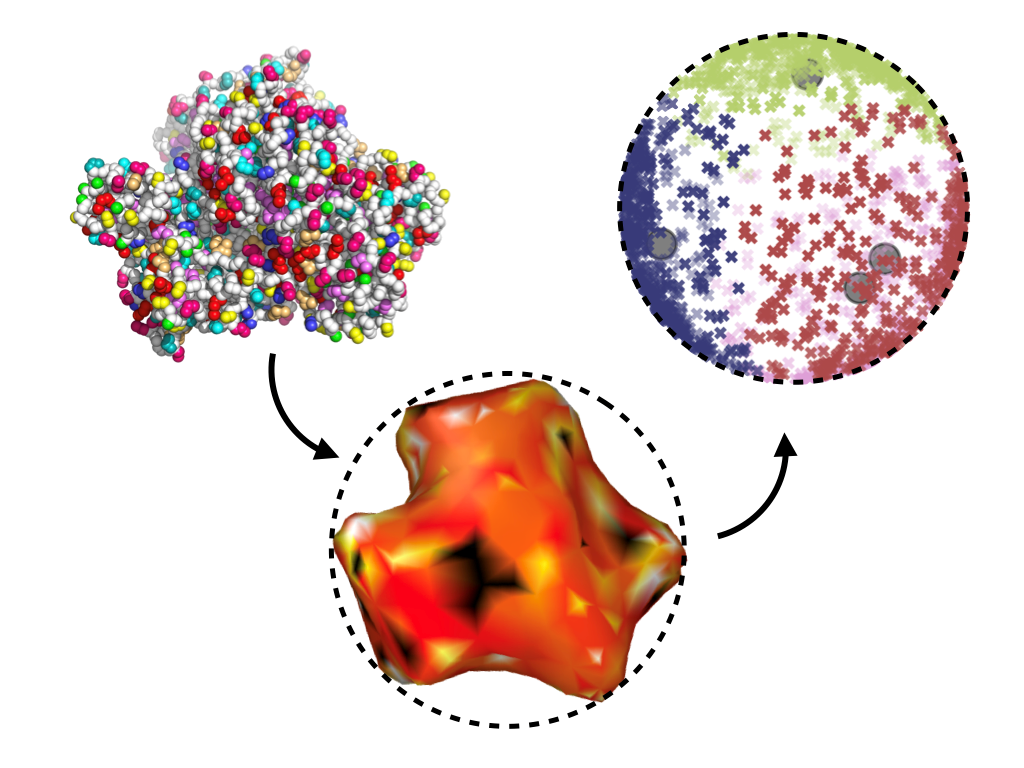
Our whole-cell models require two opposing features: computational efficiency and high-resolution molecular interactions. We developed a pipeline to map atomistic-level details to our colloidal scale whole-cell models. We extracted measurement data from the Protein Data Base (PDB)and leveraged atomic-scale detail from the structural prediction algorithm AlphaFold2. We endow our model’s finite-size Brownian particles with electrostatic, hydrophobic, topographical, and other attributes produced by amino acids within the molecular structure. Millions of these resulting proteins and complexes interact with high resolution in our models.
Protein & other colloidal gels
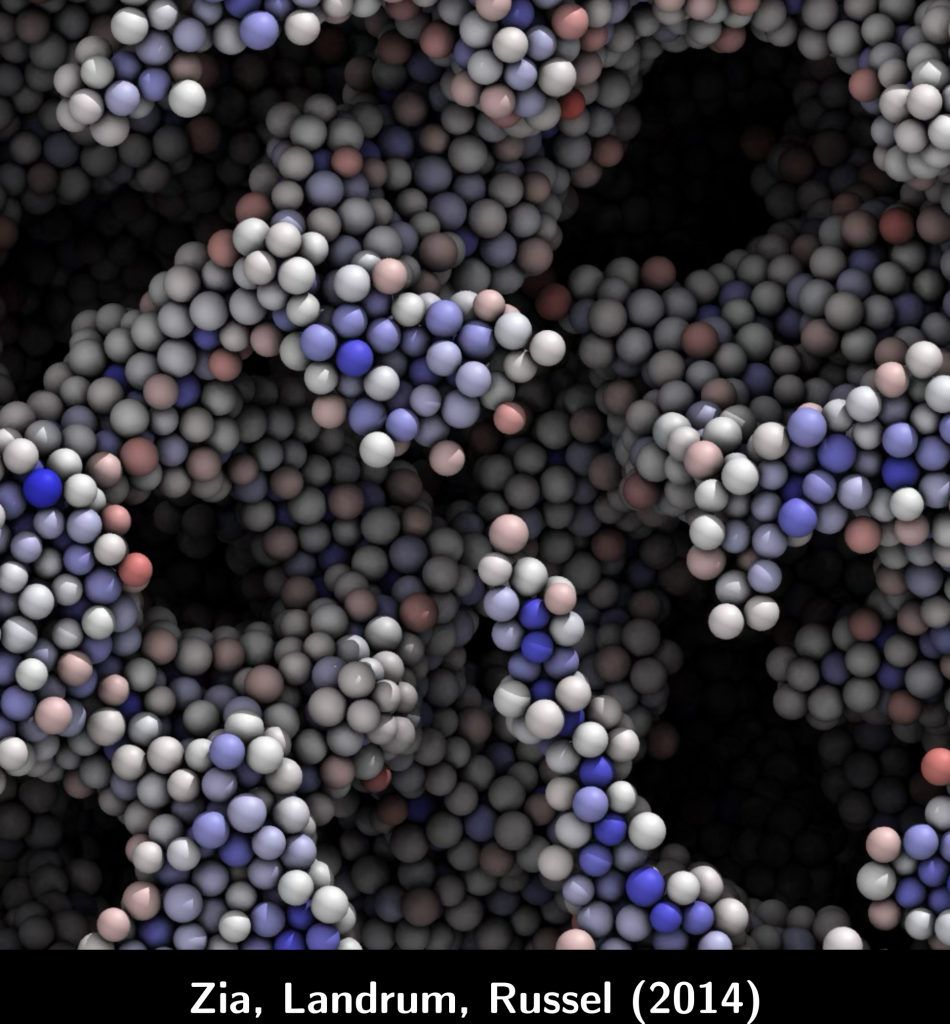
Functional efficacy, manufacturability, and shelf stability are critical characteristics of therapeutic protein solutions that are notoriously difficult to simultaneously achieve. Independent engineering of each characteristic can lead to biologically effective but undeliverable materials. To tackle this challenge, we combine multi-scale modeling and, with the Helgeson Lab at UCSB, intensified experiment workflow. We bridge sequence-level protein detail into large, efficient colloidal models of globular proteins and their interactions. This pipeline predicts suspension stability and viscoelastic properties to accelerate protein formulations and changing manufacturing and delivery conditions without compromising efficacy.
Colloidal crystals, glasses, and phase (mis)behavior
Colloidal dispersions undergo phase separation upon changing volume fraction, size composition, and interparticle forces, producing extensively reported phase envelopes. Our lab tackles challenging open questions in this area, typically focusing on phase “misbehavior”: Why colloidal gels suddenly collapse; whether colloidal glasses are truly kinetically arrested; and most recently, why dynamic simulations built to replicate monodisperse hard-sphere theory fail to produce spontaneously emerging, explicit coexistence of liquid and crystal domains, despite nearly a century of theoretical prediction and experimental confirmation.
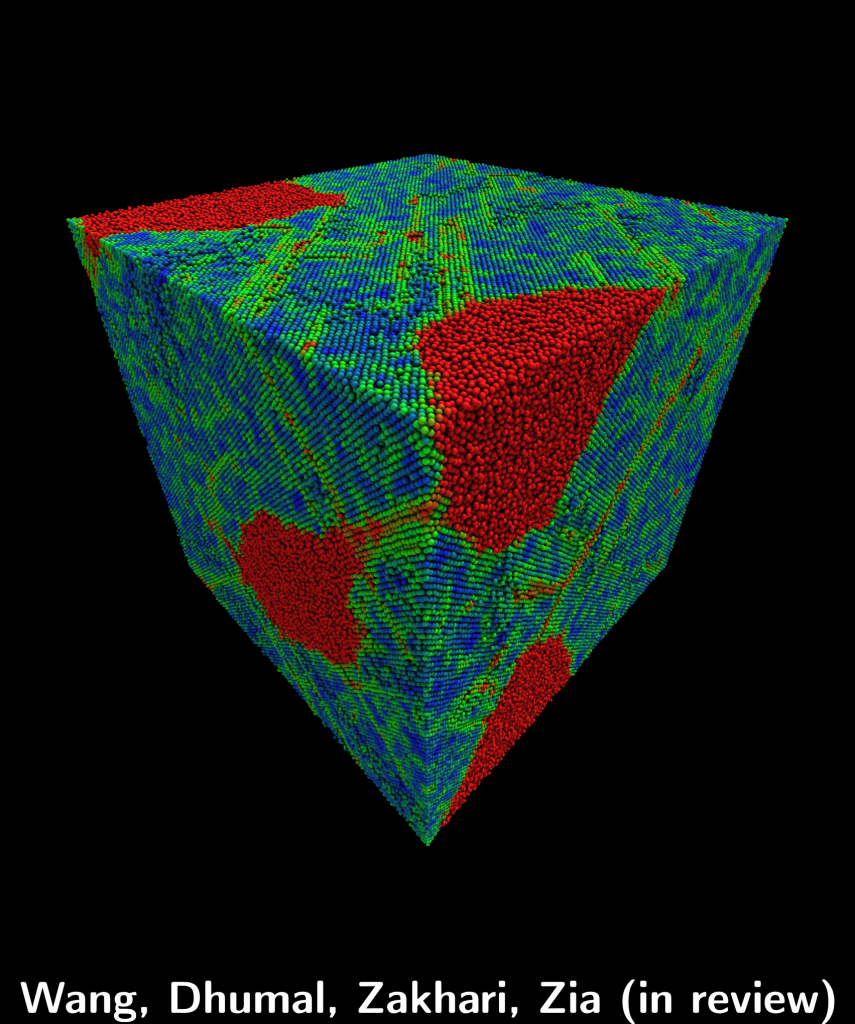